Welcome to our portfolio
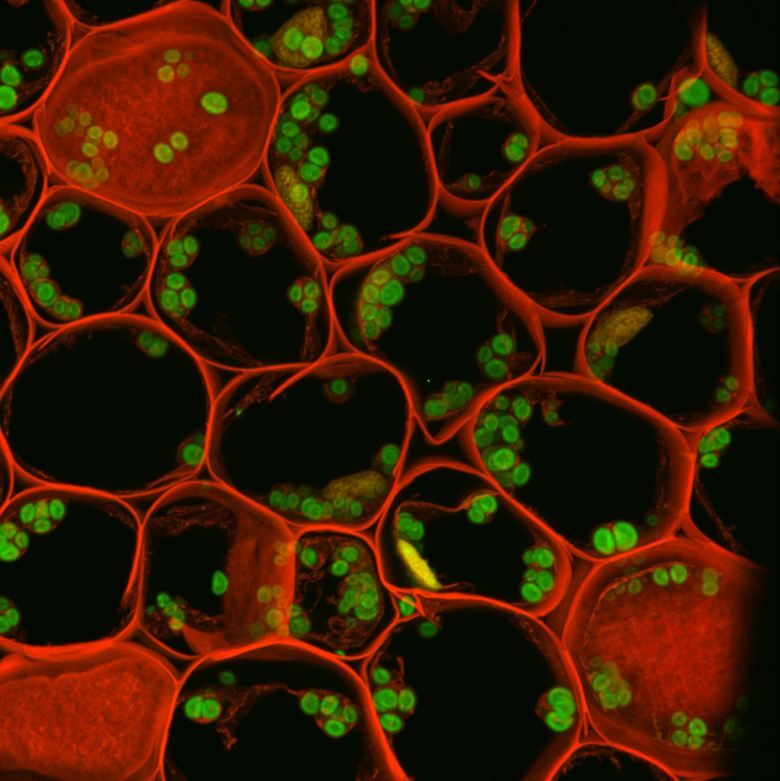
Two-photon microscopy (2P)
Multiphoton microscopy – most commonly in the form of two-photon microscopy – is a fluorescence imaging technique that allows observation of living tissue up to about one millimeter in depth. It uses pulsed red-shifted excitation laser light, which can also excite visible fluorescent dyes.
Read moreHowever, for each excitation, two (or three) photons of low energy infrared light are absorbed “simultaneously” to provide the required energy for electrons in the fluorophore to reach the excited state. Using infrared light minimizes scattering in the tissue and allows imaging deeper than is possible with common confocal microscopes. Due to the localized multiphoton absorption effect, the background signal is strongly suppressed and a pinhole is not required in front of the detector (PMTs). Both effects lead to an increased penetration depth for these microscopes. Two-photon microscopy is often used to image intravitally, and it may facilitate imaging of unlabelled tissues, using autofluorescence or SHG (second harmonic generation).
Available at: CUNI IMCF BIOCEV, CUNI VMCF, MUNI CELLIM CEITEC, IMG LM, IEM MSC, IEB IF, BUT BCF, IPHYS BIF, UPOL IMTM

Single molecule localisation microscopy (SMLM)
There are a variety of widely used implementations of single molecule localization microscopy. The unifying principle behind them is that a large series of individual images is acquired, in each of which only a small subset of the fluorescent labels in the sample are fluorescing.
Read moreThis sparse nature of the signal allows the computational calculation of the exact position of each of the emitting fluorophores. By combining the large number of images with precise locations, an image with very high resolution can be formed. Below are descriptions of two common SMLM techniques.
The fundamental principle behind stochastic optical reconstruction microscopy (STORM) is that the activated state of a photo-switchable molecule must lead to the consecutive emission of sufficient photons to enable precise localization before it enters a dark state or becomes deactivated by photobleaching. Additionally, the sparsely activated fluorescent molecules must be separated by a distance that exceeds the Abbe diffraction limit (in effect, greater than approximately 250 nanometers) to enable the parallel recording of many individual emitters, each having a distinct set of coordinates in the lateral image plane.
Photo activated localization microscopy (PALM) is a widefield fluorescence microscopy imaging methods that allow obtaining images with a resolution beyond the diffraction limit. PALM is based on collecting a large number of images each containing just a few active isolated fluorophores. The imaging sequence allows for the many emission cycles necessary to stochastically activate each fluorophore from a non-emissive state to a bright state, and back to a bleached state. During each cycle, the density of activated molecules is kept low enough that the molecular images of individual fluorophores do not typically overlap.
Available at: CUNI IMCF BIOCEV, CUNI VMCF, MUNI CELLIM CEITEC, IMG LM, UPOL IMTM
Read less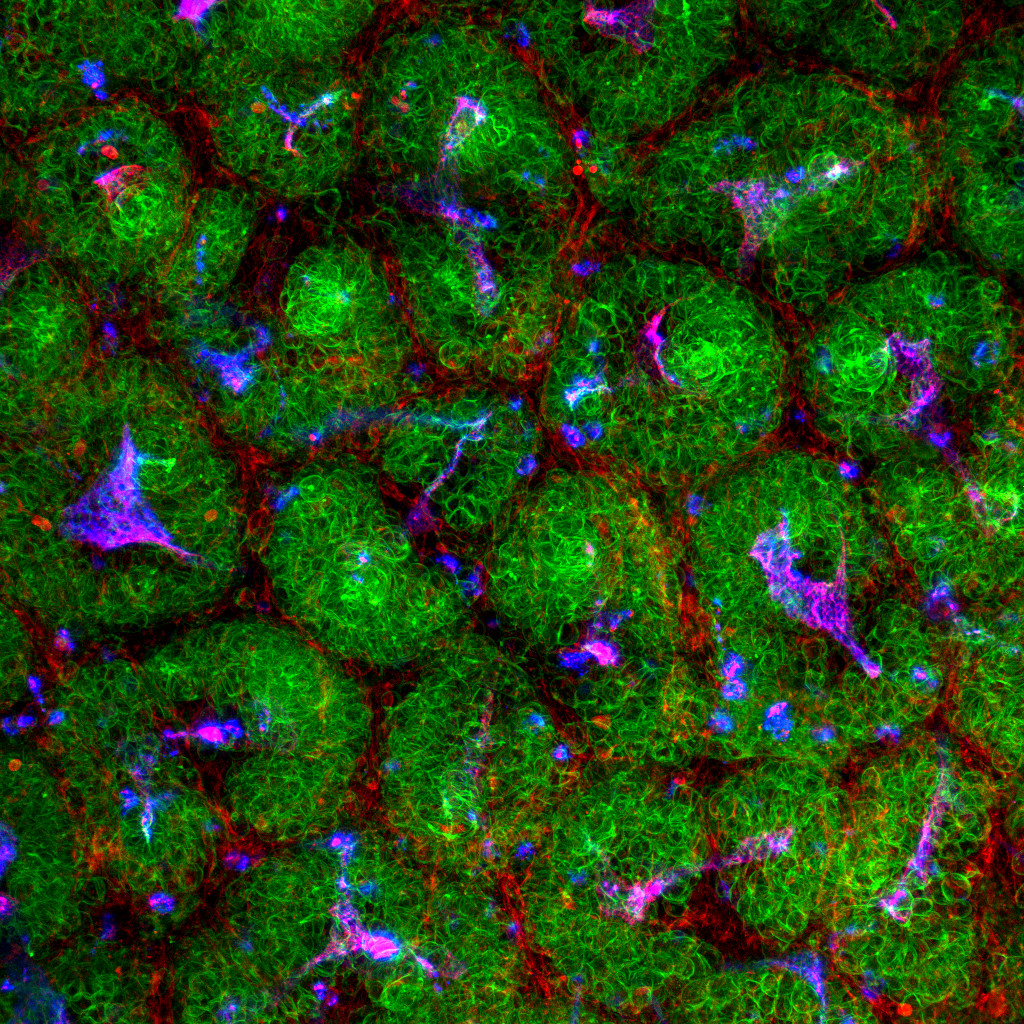
Stimulated emission depletion microscopy (STED)
Stimulated emission depletion microscopy (STED) is one of the techniques that allows super-resolution microscopy. It is similar to confocal microscopy, in that is uses laser scanning imaging, however it creates super-resolved images by the selective deactivation/depletion of fluorophores in the peripheral area of the illumination PSF, minimizing the area of illumination at the focal point, and thus enhancing the achievable resolution for a given system.
Read moreSTED can provide 3D datasets of samples, often with resolutions down to 50 nm or less.
Available at: CUNI IMCF BIOCEV, IMG LM
Read less
Light-sheet mesoscopic imaging (SPIM or dSLSM)
Light sheet microscopy is a mesoscopic imaging technology that combines optical sectioning with multiple-view imaging to observe tissues and living organisms with impressive resolution. This method is often also referred to as single plane illumination microscopy (SPIM) and many different implementations are available. Three-dimensional imaging in light-sheet-based microscopy is performed by moving the specimen through the light sheet in small steps and recording a two-dimensional image at each step.
Read moreAlternatively the light sheet can be moved through the specimen. In multiple-view imaging, the same volume inside the specimen or even the entire specimen is recorded along several angles. The resulting multiple-view information can be combined into a single image stack by data post-processing using a fusion algorithm.
Available at: MUNI CELLIM CEITEC, IMG LM, IEM MSC, IPHYS BIF
Read less
Optical projection tomography (OPT)
Optical projection tomography (OPT) is in many ways the optical equivalent of x-ray computed tomography (CT) or the medical CT scan. OPT differs in the way that it often uses ultraviolet, visible, and near-infrared photons as opposed to X-ray photons.
Read moreHowever, essential mathematics and reconstruction algorithms used for CT and OPT are similar; for example, radon transform or iterative reconstruction, based on projection data are used in both medical CT scan and OPT for 3D reconstruction.
Available at: IPHYS BIF
Read less
Coherent Anti-Stokes Raman Scattering microscopy* (CARS)
CARS microscopy is one of the label-free multiphoton imaging techniques. It allows for chemically specific imaging of biological materials without use of fluorescent labels. CARS is a three-photon nonlinear optical process where two synchronous and spatially overlapped optical beams are used to probe molecular vibrations in the sample material.
Read moreThe method is based on stimulated Raman scattering which makes CARS imaging much faster than confocal Raman mapping based on spontaneous Raman scattering (at least 100 times faster). Different experimental realizations of CARS microscopy exist. Typical is the visualization of a selected single vibrational frequency, for example the CH2 symmetric stretching mode around 2800 cm-1. Spectral scanning is realized by tuning the excitation wavelength step-by-step.
CARS has been proven useful in some very specific application fields. The CARS technique is particularly well suited for high-resolution label-free imaging of lipids due to the high concentration of carbon-hydrogen bonds in the lipid material. Lipid imaging has been applied to a great variety of samples including lipid droplets in fixed and live cell cultures, various tissue sections, and even small animals in vivo (e.g. zebrafish). In pharmaceutical applications, CARS microscopy is gaining more and more interest. Applications include visualization of chemical component distribution in dosage forms and drug carriers, dissolution and release, solid-state transformations during dissolution, and drug delivery into cells and tissues.
Available at: CUNI IMCF BIOCEV, IPHYS BIF
Read less
Quantitative Phase Imaging (QPI)
Quantitative Phase Imaging (QPI) has emerged as a valuable method for investigating cells and tissues. QPI operates on unlabelled specimens and, as such, is complementary to established fluorescence microscopy, exhibiting lower phototoxicity and no photobleaching. QPI is a label-free technique in which various methods (for example off-axis digital holography, wavefront sensing, spatial light interference, ptychography) are used to retrieve the phase information of light passing through the cell.
Read moreIn contrast to traditional qualitative label-free techniques such as phase contrast or DIC, QPI measures the absolute phase delay and is high-contrast. In 2D acquisitions the images represent quantitative maps of optical path length delays introduced by the specimen, which correspond to differences between refractive index of the cellular components and medium, and the length of the optical path within the cell. The phase delay can be directly converted into a dry mass of the cell. In 3D acquisition a spatial distribution of refractive indices is obtained, giving a three-dimensional shape of the cell and its compartments.
QPI provides an objective measure of morphology and dynamics, free of variability due to contrast agents not used. QPI data are suitable for image segmentation, making label-free cell counting and tracking easy.
The interpretation of the phase signal has proven to deliver novel parameters for studying physiological processes in living cells, such as transmembrane fluid flux, dry mass and water content changes, intracellular transport as well as tissue structure and density changes. Protein concentrations, growth and cell motility can be precisely quantified. The morphologies of cells and organelles can be established by phase tomography. Their studies provide information on the biomechanical characteristics of cell structures and membranes. These data are indicative of biomolecular activity, which can be affected by pathology.
Available at: IMG LM, BUT BCF
Read less
Second/Third Harmonics Generation (SHG/THG)
SHG is a label free technique to visualize specific structures e.g. fibrillar collagen, muscle myosin, and microtubules in vitro and in vivo. THG shows interfaces having local transitions of the refractive index e.g. between water-lipid molecules in cellular membranes and surroundings, and water-protein interfaces in protein-rich regions.
Read moreSHG and THG are not fluorescence, but coherent scattering processes instead, so there is no photobleaching and triplet state induced phototoxicity, and they are well suited especially for live sample imaging. SHG is also a very sensitive method to detect trace amounts of crystallinity e.g. in pharmaceutical materials. SHG/THG are often available in multi-photon systems which allows imaging of unlabelled samples containing aligned fibrous.
Available at: CUNI IMCF BIOCEV, IPHYS BIF
Read lessStandard methods
Commonly used and well-established methods

Laser scanning confocal microscopy (LSCM/CLSM)
Confocal laser scanning microscopy (CLSM) or laser scanning confocal [LSCM]), often colloquially referred to simply as “confocal”, is a technique for obtaining high resolution optical images with depth selectivity. The key feature of confocal microscopy is its ability to acquire in-focus images from selected depths, a process known as optical sectioning.
Read moreImages are acquired point-by-point and reconstructed with a computer. This allows three-dimensional reconstructions of topologically complex objects. However, CLSM is significantly slower than widefield or spinning-disk confocal microscopy, because images are acquired pixel-by-pixel and the laser power is concentrated on small diffraction limited spots which increases the phototoxicity load on the samples. CLSM typically requires high-laser power excitation, which can lead to phototoxicity and limited observation of live cells. This technology can be improved with image scanning mic/pixel reassignment to allow non-diffraction limited images which provide resolutions 100-200nm.
Available at: CUNI IMCF BIOCEV, CUNI VMCF, MUNI CELLIM CEITEC, IMG LM, IEM MSC, IEB IF, BUT BCF, IPHYS BIF, UPOL IMTM
Read less
Spinning disc confocal microscopy (SDCM)
Spinning disc confocal microscopy is one of the solutions for routine and high-performance fluorescence live-cell imaging applications. SDCM uses a series of moving pinholes on a disc to scan spots of light, in combination with a high-sensitivity camera to acquire instantaneous optical slices.
Read moreSince a series of pinholes scans an area in parallel, each pinhole is allowed to hover over a specific area for a longer amount of time than on laser scanning confocals (CLSM) thereby reducing the excitation energy needed to illuminate a sample . Furthermore, cameras (often EMCCDs or sCMOS), typically have quantum efficiencies 2-3x higher than PMTs, so much less laser excitation energy is necessary and that reduces photo-toxicity and photo-bleaching of a sample, making it the preferred system for imaging live cells or organisms when optical slicing is necessary. However, optical sectioning in depth is more limited than in CLSM. This technology can be improved with image scanning mic/pixel reassignment to allow non-diffraction limited images which provide resolutions 100-200nm.
Available at: IMG LM, IEM MSC, IEB IF, IPHYS BIF, UPOL IMTM
Read less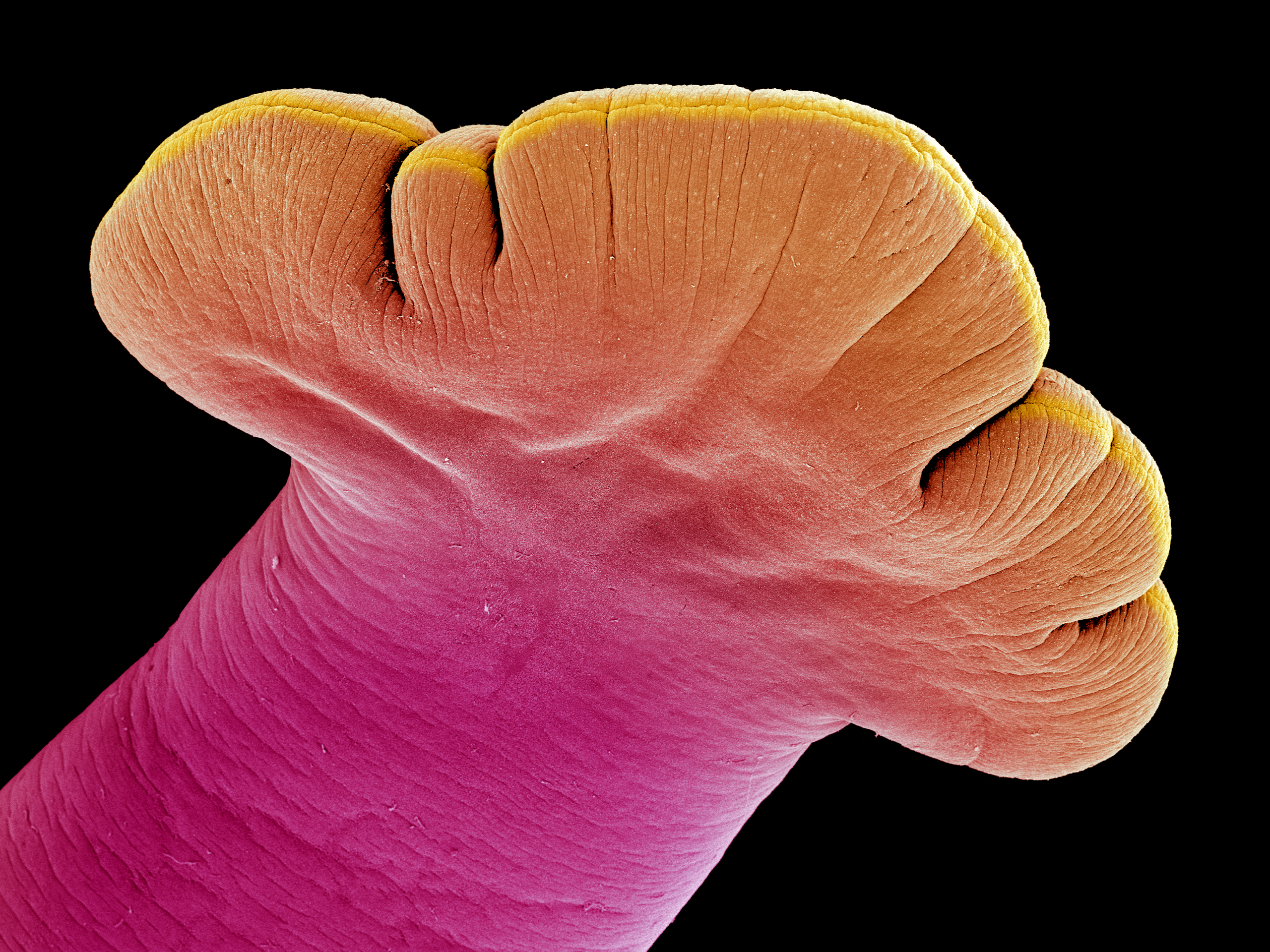
Structured illumination microscopy (SIM)
SIM belongs to the family of super-resolution microscopy techniques that allow to acquire images with higher spatial resolution than with conventional fluorescence microscopy. SIM is a camera based widefield fluorescence microscope employing a patterned illumination, e.g. stripes, to excite the fluorescence in the sample.
Read moreThe diffraction limited structured illumination pattern is the key element that allows to extract higher frequencies from the sample and thus achieve the improved spatial resolution. To obtain one super-resolution image, several images with shifted and rotated illumination patterns projected on the sample are acquired. The set of acquired images is then mathematically processed to reconstruct the super-resolution image. The reachable resolution improvement, both laterally and axially, is twice as better as with conventional widefield microscopy for the same wavelength. Data acquisition for one super-resolution SIM image takes from hundreds of milliseconds to seconds.
SIM is a versatile super-resolution technique used for a detailed imaging of cellular structures within cell monolayers or thin tissue sections. The strength of the method lies in the compatibility with a high number of standard fluorophores allowing relatively simple multi-color imaging, and in live-cell imaging friendliness in terms of acquisition speed and used laser powers (phototoxicity). The downside of SIM compared to other super-resolution techniques (STED, SMLM) is a moderate resolution improvement and proneness to image artifacts formation.
Available at: CUNI VMCF, MUNI CELLIM CEITEC, IMG LM, IEB IF, UPOL IMTM
Read less
Total internal reflection fluorescence microscopy (TIRF)
Total internal reflection fluorescence microscopy (TIRF) is a microscopy technique with which a thin region of the cell, usually less than 200nm can be observed. A TIRF microscope uses an evanescent wave to selectively illuminate and excite fluorophores in a restricted region of the specimen immediately adjacent to the glass-water interface.
Read moreThe evanescent wave is generated only when the incident light is totally internally reflected at the glass-water interface. The evanescent electromagnetic field decays exponentially from the interface, and thus penetrates to a depth of only approximately 100 nm into the sample medium. Thus the TIRF microscope enables a selective visualization of surface regions such as the basal plasma membrane (which are about 7.5 nm thick) of cells. This technique is often used also for observing molecular dynamics in vitro, or study the details of cell locomotion or adhesion to substrata.
Available at: CUNI IMCF BIOCEV, CUNI VMCF, IMG LM
Read less
Fluorescence (cross)-correlation spectroscopy (FCS/FCCS)
Fluorescence correlation spectroscopy (FCS) is a correlation analysis of fluctuations in the fluorescence intensity. The analysis provides information on physical parameters of the fluorescent particles (molecules) in solution, such as concentration, average fluorescence intensity and diffusion speed.
Read moreBy following changes on these parameters it is possible to study binding events of the molecules or even conformational changes on them.
Fluorescence cross-correlation spectroscopy (FCCS) extends the FCS procedure in that it looks at the correlation between different colors (cross-correlation) rather than just the same color (auto-correlation). In other words, coincident green and red intensity fluctuations correlate if green and red labeled particles are moving together. As a result, FCCS provides a highly sensitive measurement of molecular interactions independent of diffusion rate.
Available at: CUNI IMCF BIOCEV, CUNI VMCF
Read less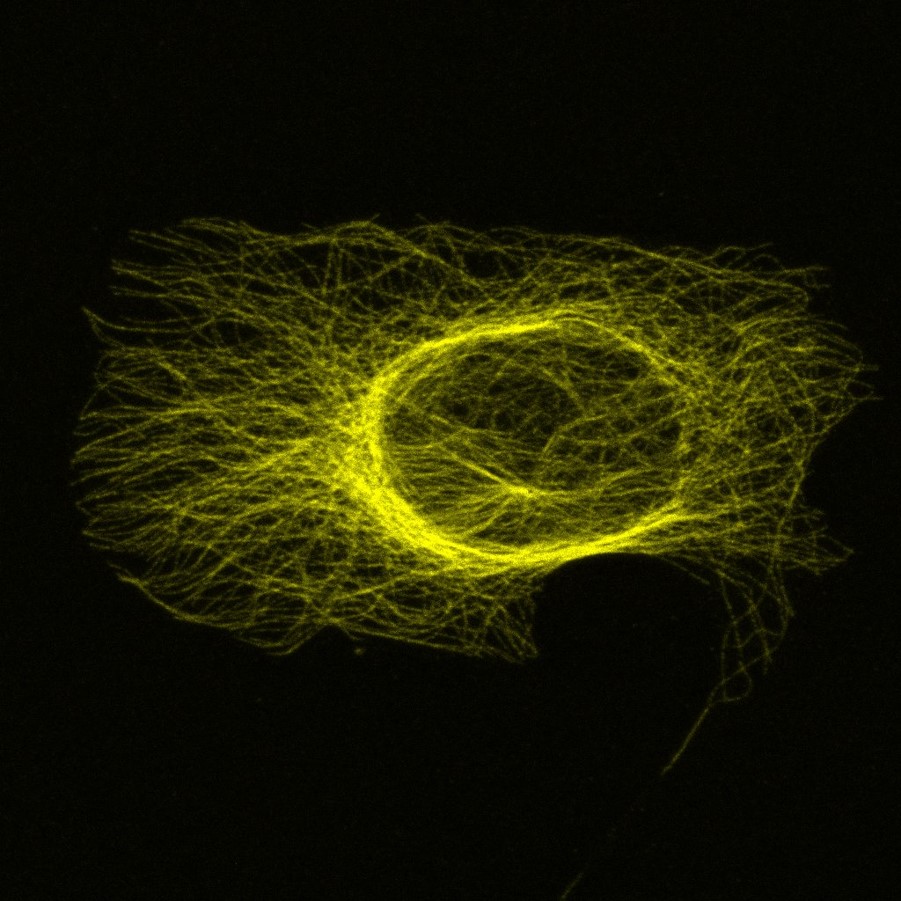
Fluorescence Resonance Energy Transfer (FRET)
Fluorescence resonance energy transfer (FRET) is a mechanism describing energy transfer between two light-sensitive molecules (chromophores). A donor chromophore, initially in its electronic excited state, may transfer energy to an acceptor chromophore through non-radiative dipole-dipole coupling.
Read moreThe efficiency of this energy transfer is inversely proportional to the sixth power of the distance between donor and acceptor, making FRET extremely sensitive to small changes in distance and therefore an excellent reporter on molecule proximity and interaction.
Available at: CUNI VMCF, IMG LM
Read less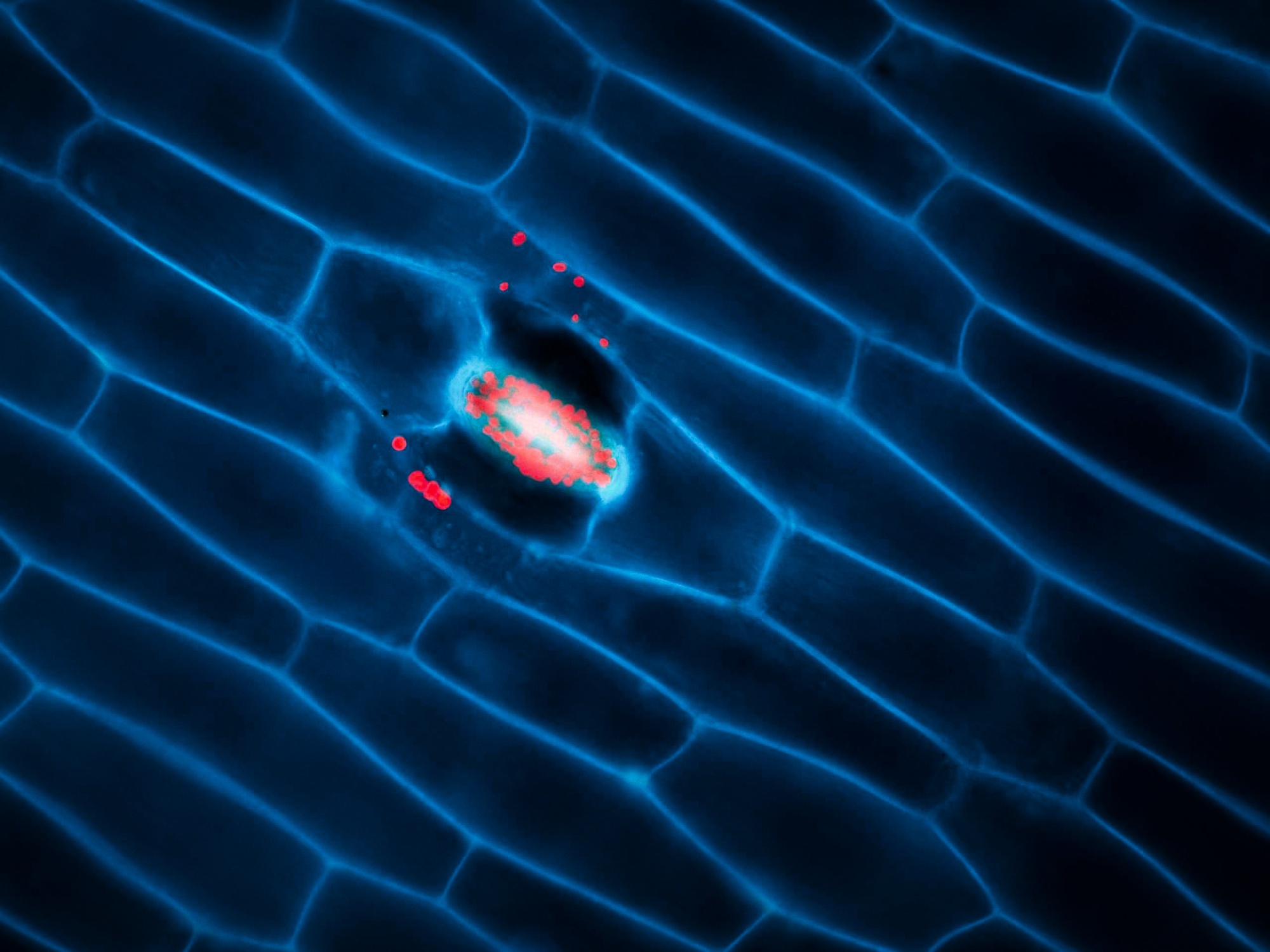
Fluorescence Recovery after Photobleaching (FRAP)
Fluorescence recovery after photobleaching (FRAP) denotes an optical technique capable of quantifying the two dimensional lateral diffusion of a molecularly thin film containing fluorescently labeled probes.
Read moreThis technique is very useful in biological studies of cell membrane diffusion and protein binding as it not only reports on the diffusion rates of mobile fractions of molecules but also provides information about the proportion of immobile molecules. In addition, surface deposition of a fluorescent phospholipid bilayer (or monolayer) allows the characterization of hydrophilic (or hydrophobic) surfaces in terms of surface structure and free energy.
Available at: CUNI VMCF, IMG LM, IEM MSC
Read less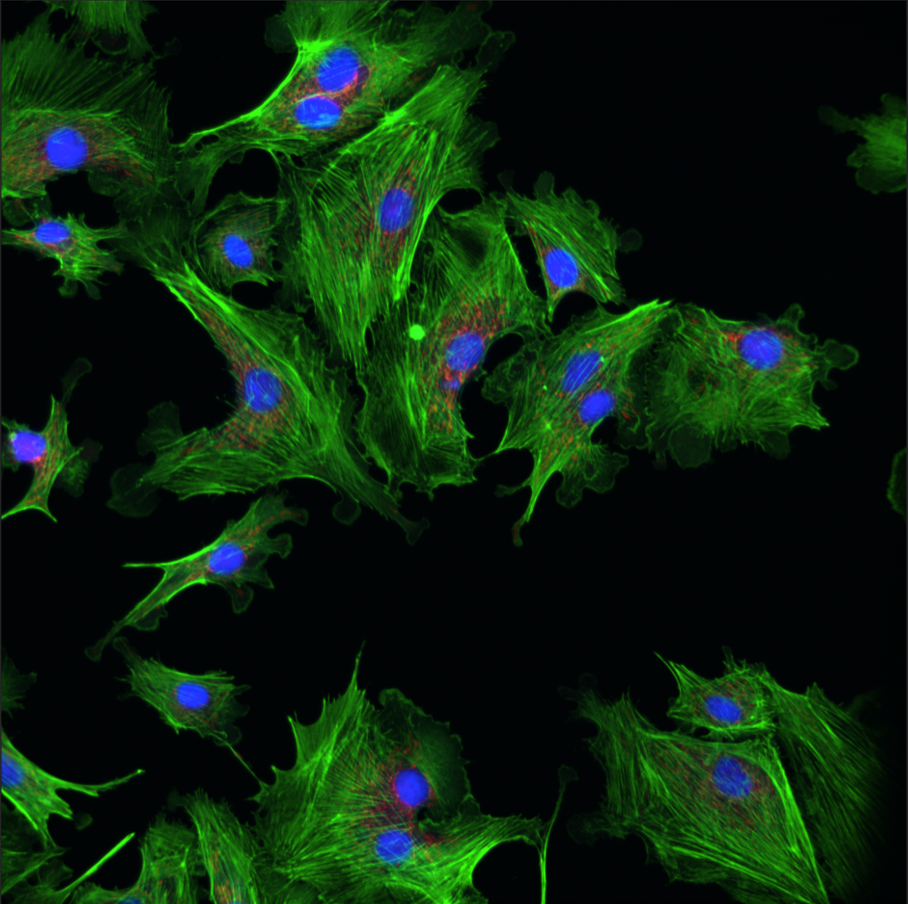
Fluorescence Lifetime Imaging (FLIM)
Fluorescence-lifetime imaging microscopy (FLIM) is an imaging technique for producing an image based on differences in the fluorescence-lifetime rather than its intensity.
Read moreBy quantifying variations in the exponential decay rate of the fluorescence from a fluorescent sample (fluorescence-lifetime) it is possible to report on molecule proximity, pH changes and even polarity. It can be used as an imaging technique in confocal microscopy, two-photon excitation microscopy and multiphoton tomography. Since the fluorescence-lifetime is insensitive to changes in fluorophore intensity or concentration, it is the most quantitatively precise technique to report on fluoresce resonance energy transfer (FRET).
Available at: CUNI IMCF BIOCEV, CUNI VMCF, IMG LM
Read less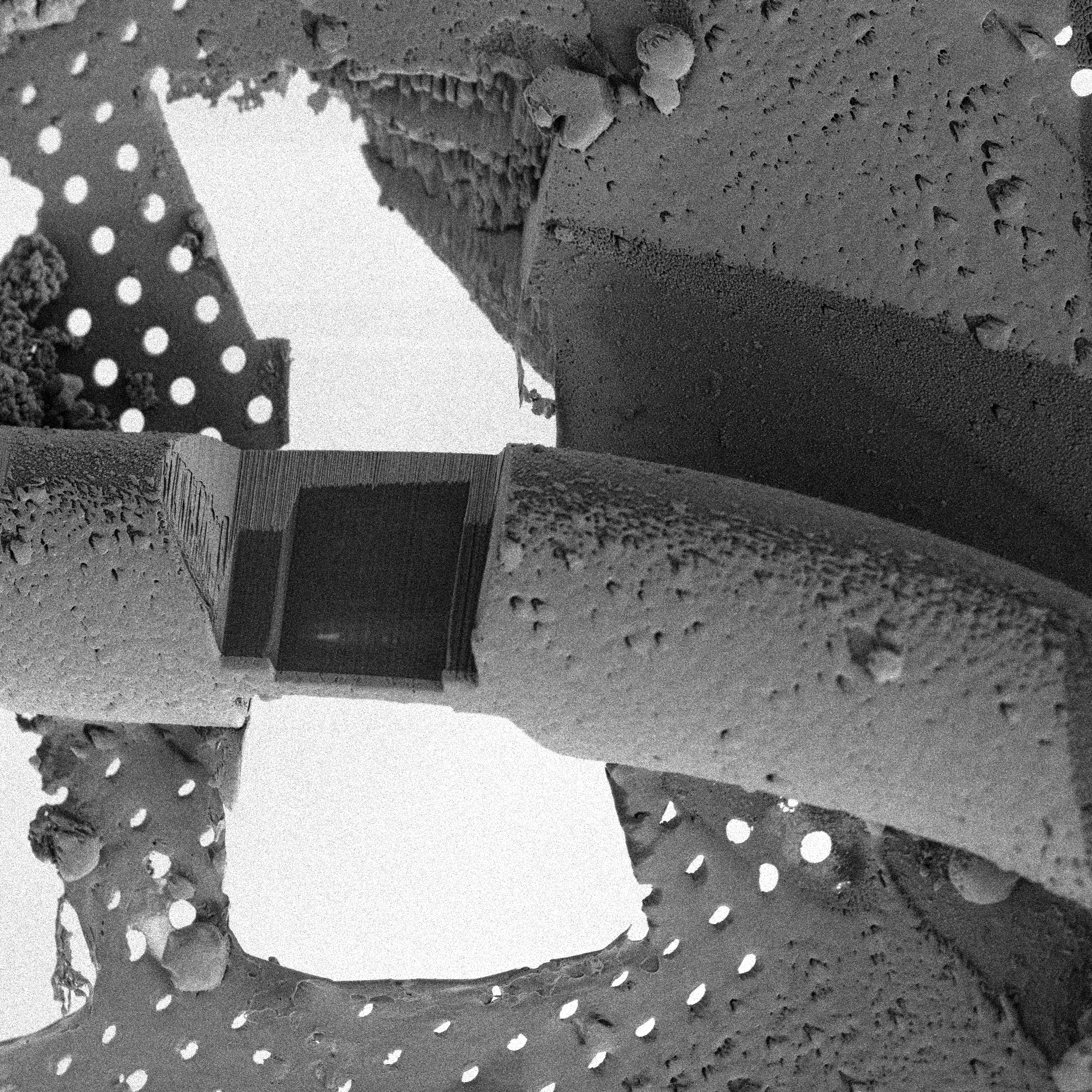
Conventional electron microscopy (SEM/TEM)
Conventional EM enables the visualisation of cell structures at the best resolution available. The specimen preparation techniques for TEM usually include chemical methods (fixation, postfixation, dehydration, resin infiltration, embedding, ultrathin sectioning, and contrasting) as well as advanced high-pressure freezing/freeze substitution techniques.
Read moreSpecimens for SEM are usually critical-point dried and coated with metal such as gold, platinum to make their surfaces conductive. Also other preparation techniques are available.
Available at:
▪ TEM (BC LEM, IMG EM, CUNI IMCF BIOCEV, ISI EM,CUNI VMCF)
▪ SEM (BC LEM, IMG EM, CUNI IMCF BIOCEV, ISI EM,CUNI VMCF)
Read less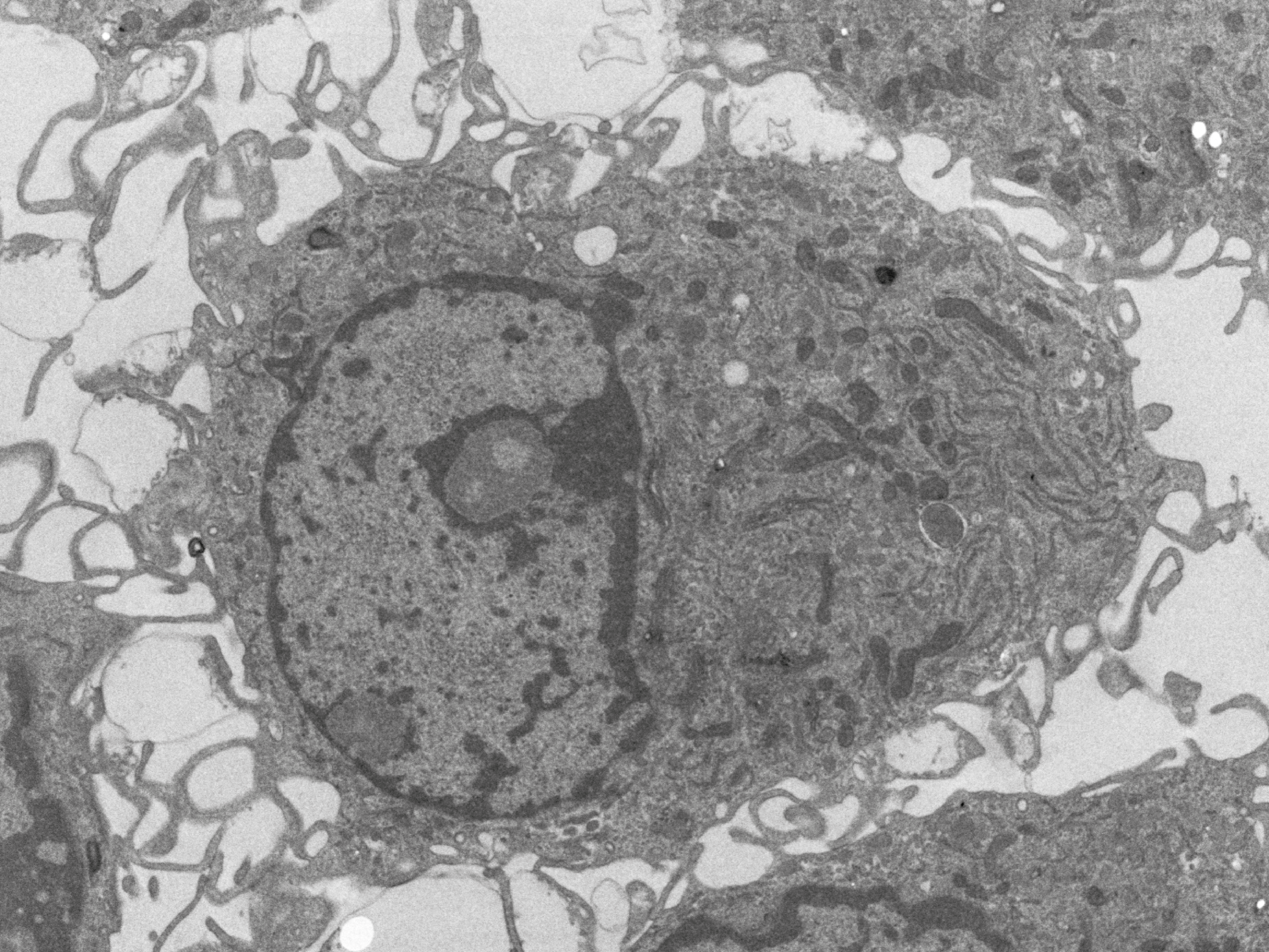
Volume electron microscopy (vEM)
vEM is used to image the spatial organisation of structures of biomolecules, cells, tissues and organisms. vEM includes both TEM and SEM-based technologies, in which a series of images are produced, combined and finally a digital representation of the sample volume is formed.
Read moreIndividual images of the sample are acquired either at different inclinations (TEM tomography), by imaging serial sections (array tomography, serial-section-TEM) or by imaging the surface of the sample block after ion milling (FIB-SEM) or slicing off a thin layer (SBF-SEM). Services are provided including data storage, image analysis including AI assisted and 3D modelling.
Facilities offering these technologies:
▪ TEM-tomography (BC LEM, IMG EM, CUNI IMCF BIOCEV, CUNI VMCF)
▪ FIB-SEM (CUNI IMCF BIOCEV)
▪ SBF-SEM (BC LEM)
▪ Array tomography (CUNI VMCF, BC LEM)
▪ Serial-section TEM (BC LEM, IMG EM, CUNI IMCF BIOCEV, CUNI VMCF)
Read less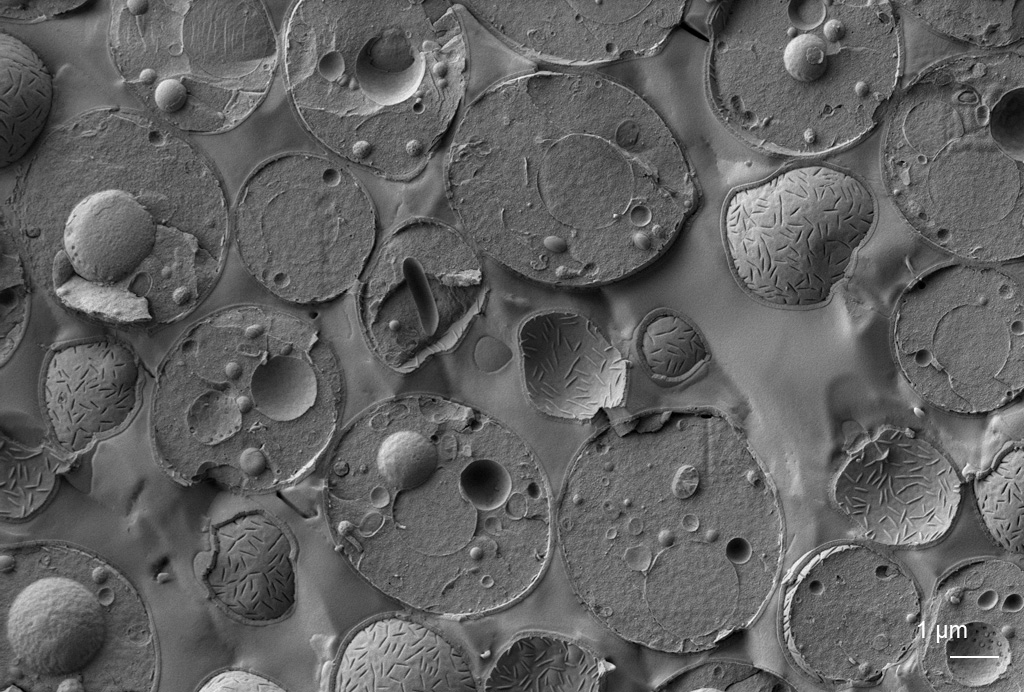
Cryo-electron microscopy
CryoEM is an essential tool for structural biology and increasingly also for cell biology. Vitrified samples are imaged using the direct electron detector in TEM (cryoTEM). Using subsequent image analysis, the structures of macromolecules can be studied in vitro for purified macromolecules (SPA) or in situ for assemblies in a cellular context, where lamellae produced by focused ion beam milling are observed cryo electron tomography (cryo-ET).
Read moreCryo-SEM enables observation of surface topography and can be combined with ion milling (FIB-SEM), freeze fracturing, EDX and Raman analysis and fluorescence microscopy (cryo-CLEM). Cryo-CLEM can be used to localise regions of interest or determine where fluorescently labelled structures are present in the frozen sample.
Facilities offering these technologies:
▪ cryoTEM (BC LEM, IMG EM, CUNI IMCF BIOCEV)
▪ cryoFIB-SEM (IMG EM)
▪ cryo-SPA (BC LEM, CUNI IMCF BIOCEV)
▪ cryo-ET (BC LEM, IMG EM, CUNI IMCF BIOCEV)
▪ cryo-SEM (BC LEM, ISI EM, CUNI IMCF BIOCEV)
▪ cryo-CLEM (IMG EM, CUNI IMCF BIOCEV)
Read less
Immunoelectron microscopy
Immunoelectron microscopy is a powerful technique for detecting and locating antigens in their cellular context. Available methodologies combining immuno/affinity labelling are negative staining, Tokuyasu technique, embedding in acrylic resins, freeze-fractured replica labelling, array-tomography and pre-embedding labelling using different markers as well as correlative light electron microscopy techniques.
Available at:
▪ Tokuyasu technique (BC LEM, IMG EM, CUNI IMCF BIOCEV, CUNI VMCF)
▪ Immunogold/negative staining (all)
▪ Pre-embedding immunoEM (all)
▪ Post-embedding immunoEM (all)
▪ Array tomography (CUNI VMCF, BC LEM)
Read less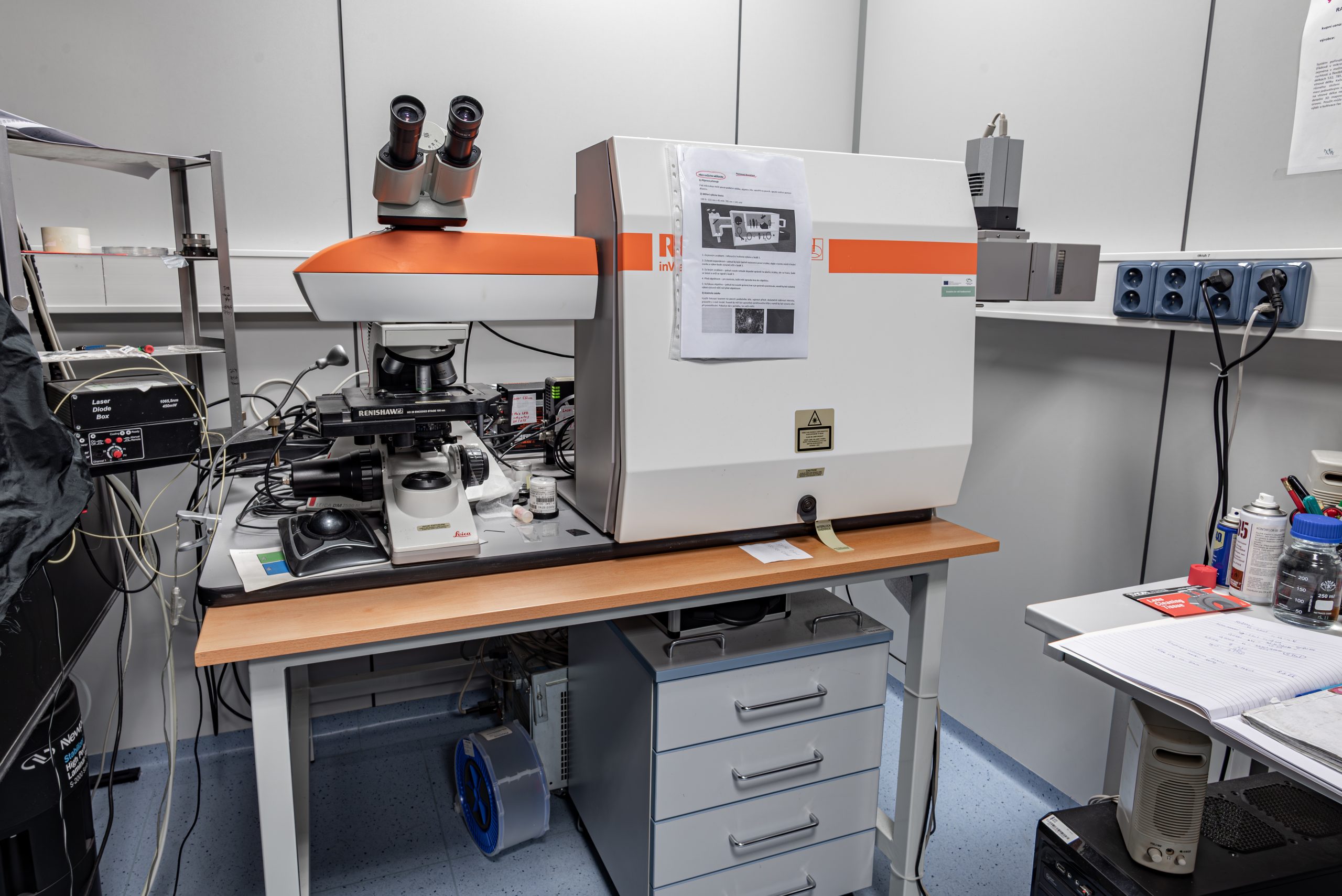
Optical projection tomography (OPT)
In addition to imaging the structure at high resolution, the composition of the sample can also be determined using EDS/EDXS, which relies on the X-rays emitted by the elements due to their interaction with the primary beam electrons. Elemental composition can be detected in TEM, SEM and FIB-SEM.
Read moreChemical composition can be also obtained using correlative SEM – Raman imaging optionally at cryogenic temperatures. Microcrystalline compounds can be characterised by electron diffraction.
Available at:
▪ SEM-EDX (EM ISI, CUNI IMCF BIOCEV)
▪ RAMAN-SEM (BC LEM)
▪ TEM-EDX (IMG EM)
▪ RAMAN microscopy (EM ISI)
▪ Electron diffraction (EM ISI, IMG EM)
Read less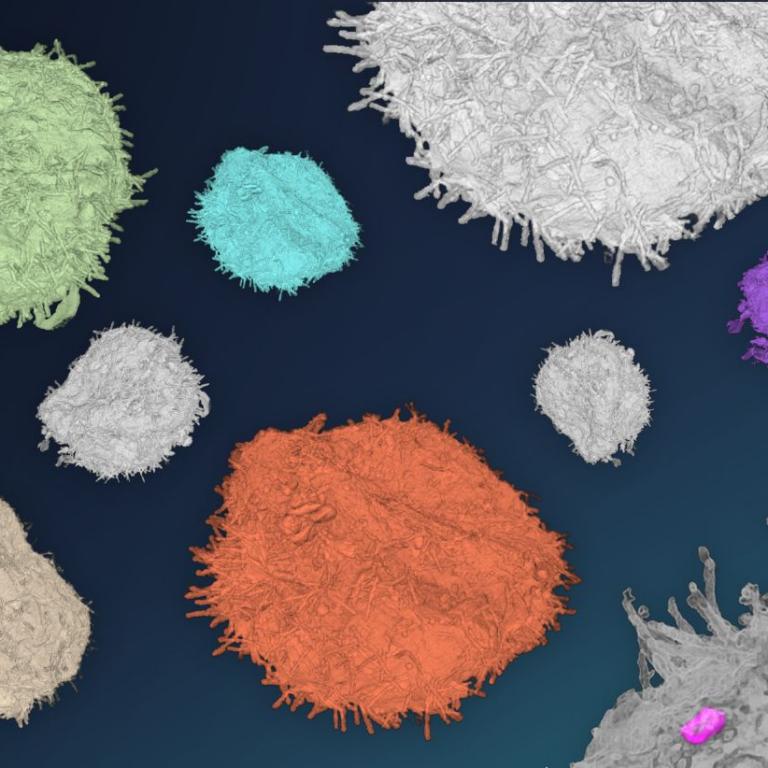
Correlative light and electron microscopy
Correlative light electron microscopy (CLEM) combines images obtained from the same areas of interest using both light and electron microscopy. The aims are to identify specific structures in EM sample volume size, locate rare structures, capture dynamic events, to get information of the cellular context surrounding the specific structure, etc.
Available approaches may combine live imaging, fluorescence imaging (wide-field, confocal, light sheet), observation of vitrified samples using cryo-fluorescence and cryo-EM, “on-section” techniques, TEM, SEM, volume EM techniques, immuno-EM, etc.
Available at:
▪ CLEM on Tokuyasu sections (BC LEM, CUNI IMCF BIOCEV)
▪ CLEM from live cells to 3D plastic embedded samples (IMG EM, BC LEM, CUNI IMCF BIOCEV)
▪ cryo-CLEM (CUNI IMCF BIOCEV, IMG EM)
Read lessPreclinical imaging
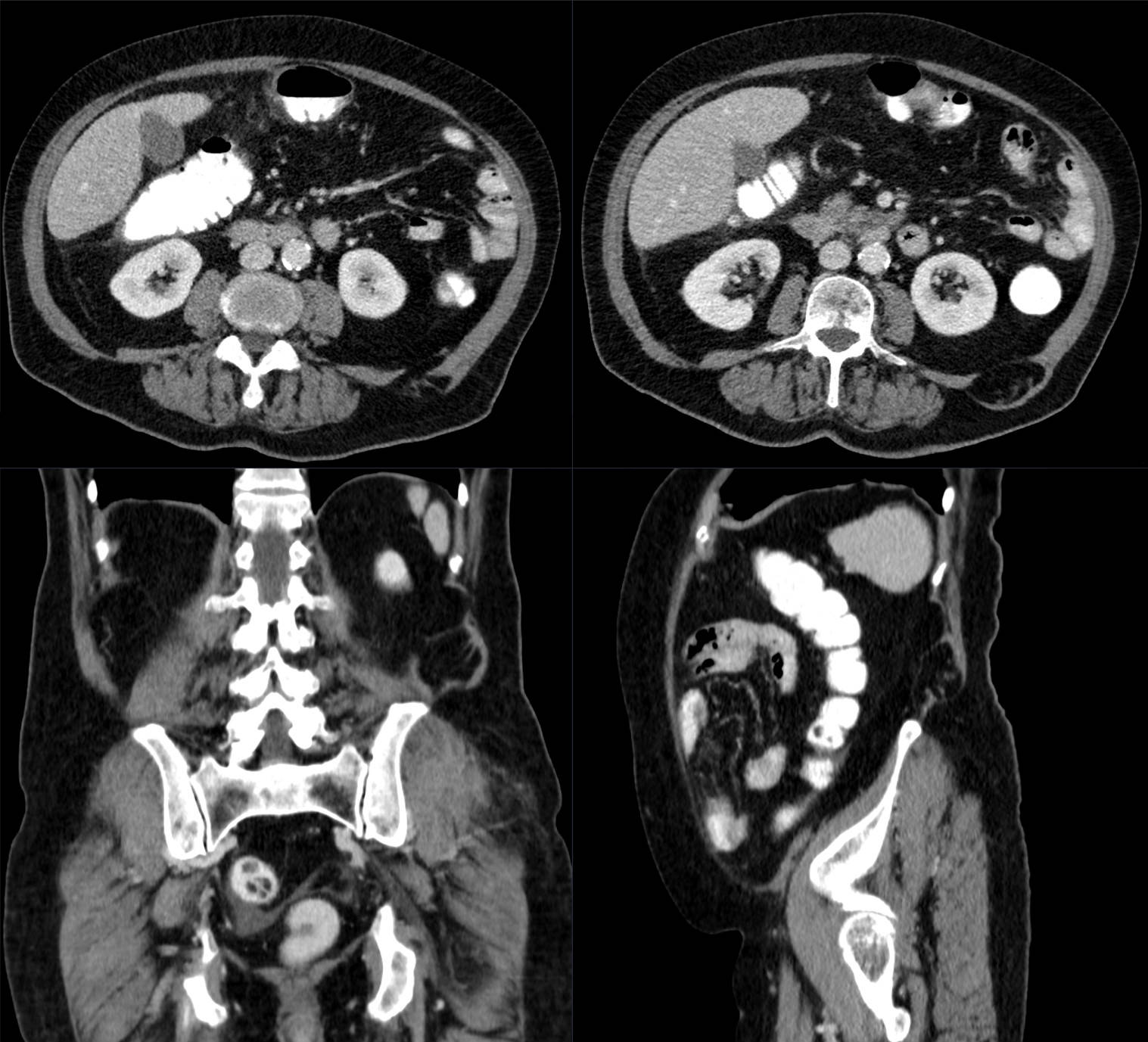
Small animal Computed Tomography (CT)
X-rays Computed Tomography (CT) is based on reconstruction of projections (X-ray images taken from different angles), which provides tomographic anatomical information of measured tissue.
Read moreThe image contrast can be enhanced with CT contrast agents. The spatial resolution of animal CT is typically higher with respect to clinical CT.
Available at: CUNI CAPI
Read less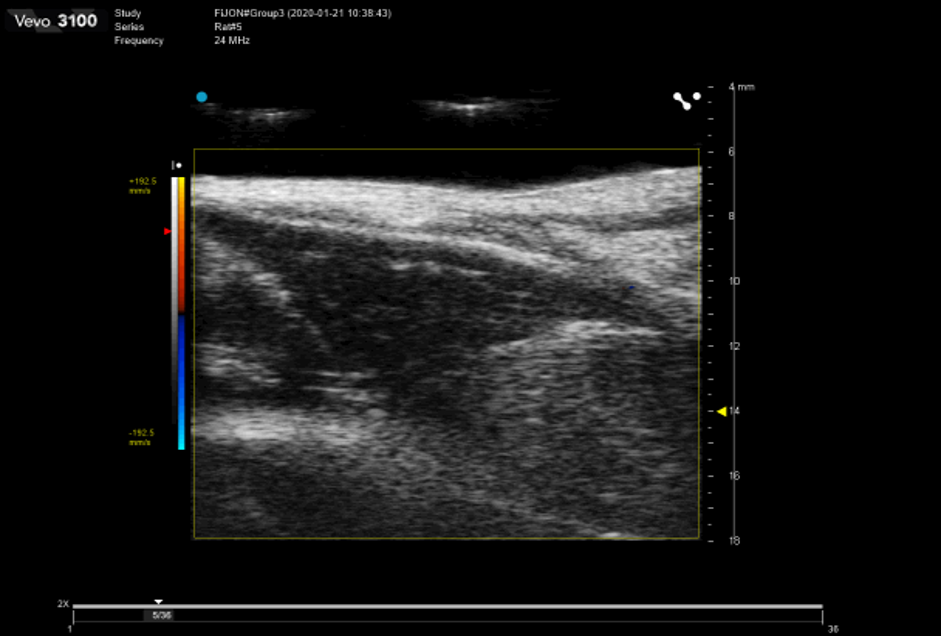
Small animal Positron Emission Tomography (PET)
A functional imaging technique that uses radioactive substances known as radiotracers to visualize and measure changes in metabolic processes, and in other physiological activities including blood flow, regional chemical composition, and absorption.
Read moreDifferent tracers are used for various imaging purposes, depending on the target process within the body. It can be used in oncology, neurology, cardiology and other medical research topics.
Available at: CUNI CAPI
Read less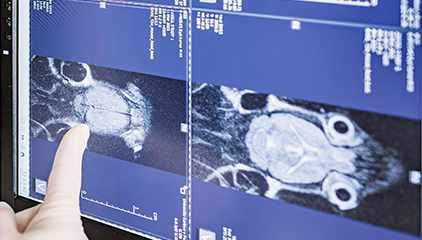
Small animal Single-Photon Emission Computed Tomography (SPECT)
o Single-photon emission computed tomography (SPECT) is a nuclear medicine tomographic imaging technique using gamma rays produced by radiotracer (gamma-emitting radioisotope) injected to observed tissue.
Read moreIt is very similar to conventional nuclear medicine planar imaging using a gamma camera but is able to provide true 3D (tomographic) information. SPECT can be helpful, for instance, in tumor imaging, infection imaging, thyroid imaging or bone scintigraphy.
Available at: CUNI CAPI
Read less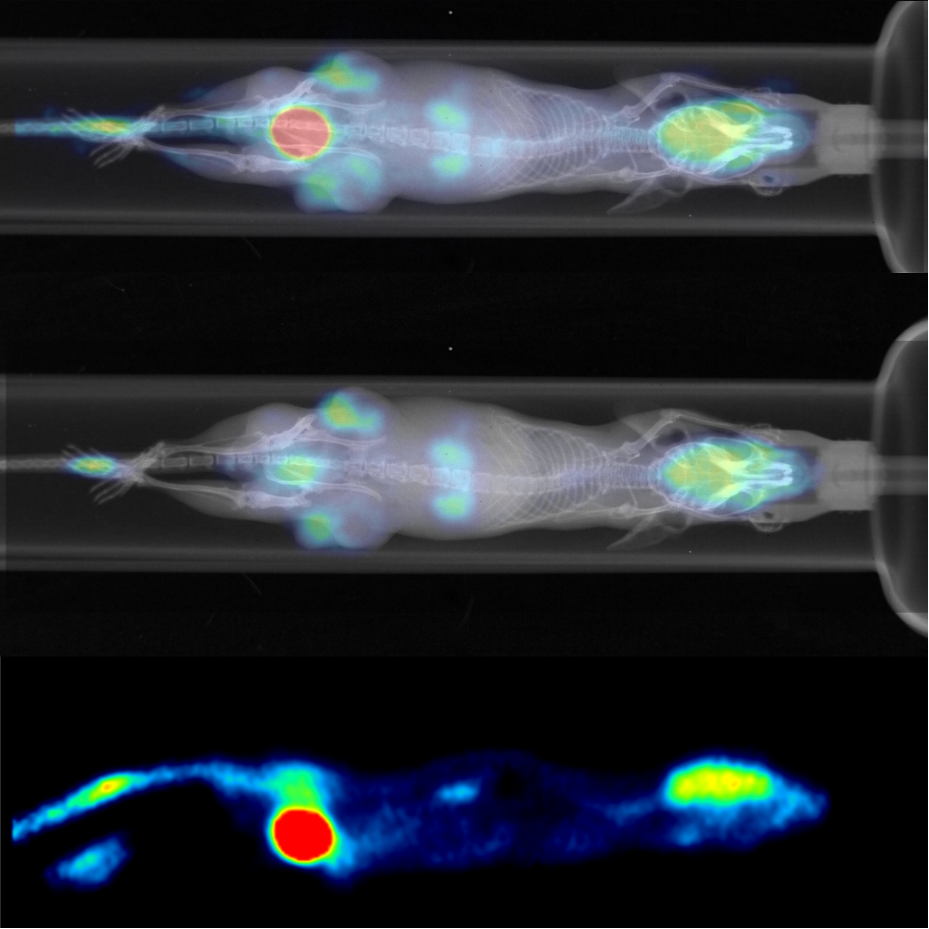
Small animal Single-Photon Emission Computed Tomography (SPECT)
Magnetic resonance imaging (MRI) is a non invasive imaging technique used to obtain 2D/3D in vivo images representing anatomy and physiological processes (e.g. dynamic perfusion or dynamic changes in blood oxygenation for functional brain mapping), with high spatial and temporal resolution. MRI scanners use strong magnetic fields (for animal studies typically 4.7T to 9.4T or even stronger), radiofrequency pulses, and field gradients to generate images of soft tissues throughout the body.
Read moreImages can be enhanced with contrast agents affecting the properties of the tissue (e.g. changing relaxation times). Magnetic Resonance Spectroscopy (MRS) can be used to complement MRI in the characterization of tissues. In preclinical imaging, animal models of various pathologies, from tumors to cardiovascular, neurological or metabolic disorders, are commonly used to monitor disease development and to test the efficacy of therapeutic treatments, to develop novel diagnostic tools etc. Several quantitative imaging biomarkers can be used to monitor disease progression over time.
MRI scanners can be used in many regimes providing several different MRI techniques. For instance: Anatomical MRI, Relaxometry, Diffusion MRI, Perfusion MRI, Functional MRI, Susceptibility weighted imaging and mapping (SWI/SWM), Chemical Exchange Saturation Transfer (CEST) and Magnetization Transfer (MT), etc.
Available at: ISI MR, CUNI CAPI
Read less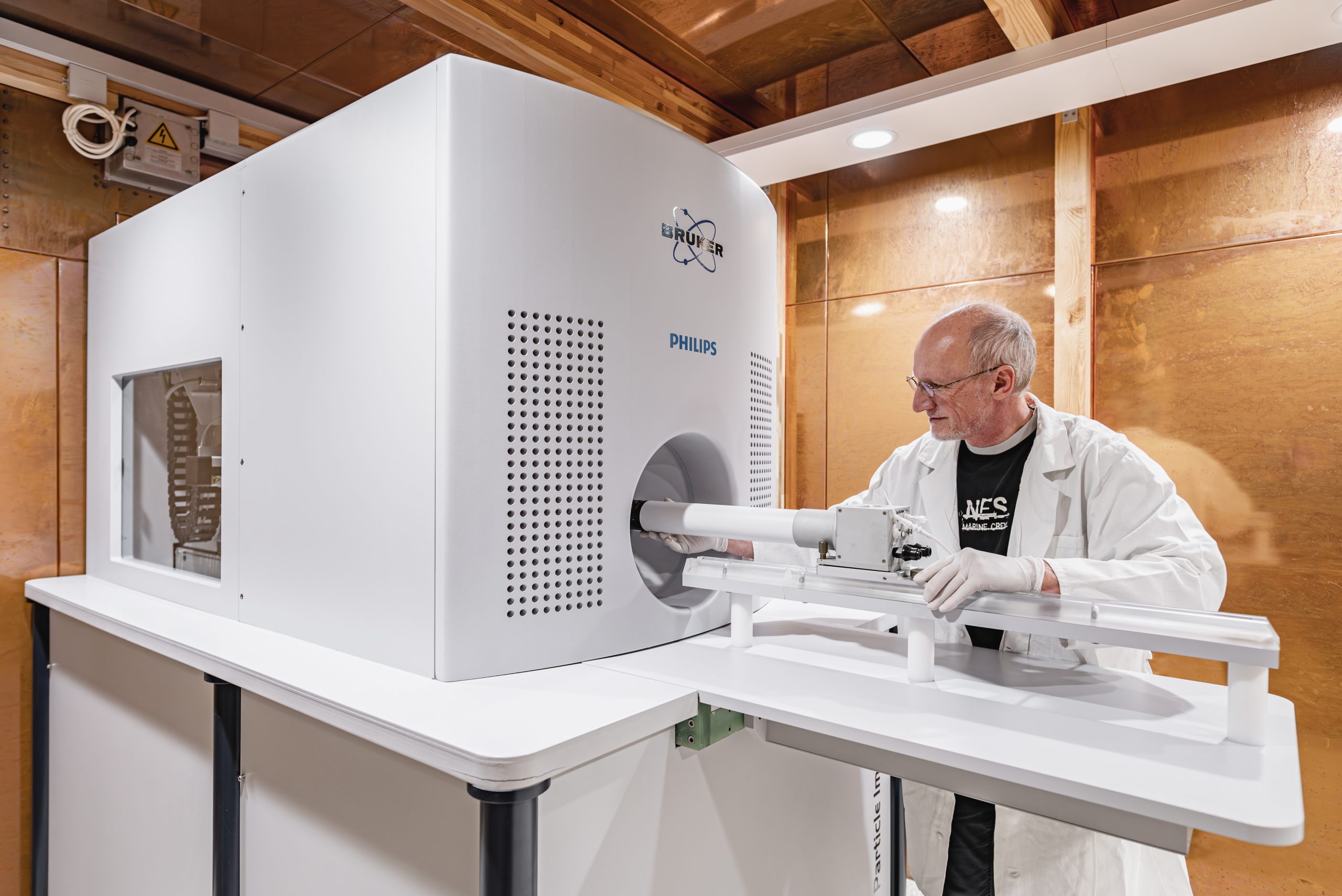
Small animal Magnetic Particle Imaging (MPI)
Magnetic particle imaging is a tomographic method based on detection of nonlinear response of superparamagnetic tracers (usually superparamagnetic Iron Oxide, SPIO) to alternating magnetic fields.
Read moreThe method detects the tracer only (i.e., it is a hot spot imaging). Therefore MPI images have no background signal, but require colocalization with an anatomical imaging method, such as MRI or CT. At moderate spatial resolution, MPI provides high sensitivity and superb temporal resolution. Current main applications are in the preclinical field and include biodistribution of nanoparticles, cell tracking, detection of nanoparticles deployed for thermoablation.
Available at: CUNI CAPI
Read less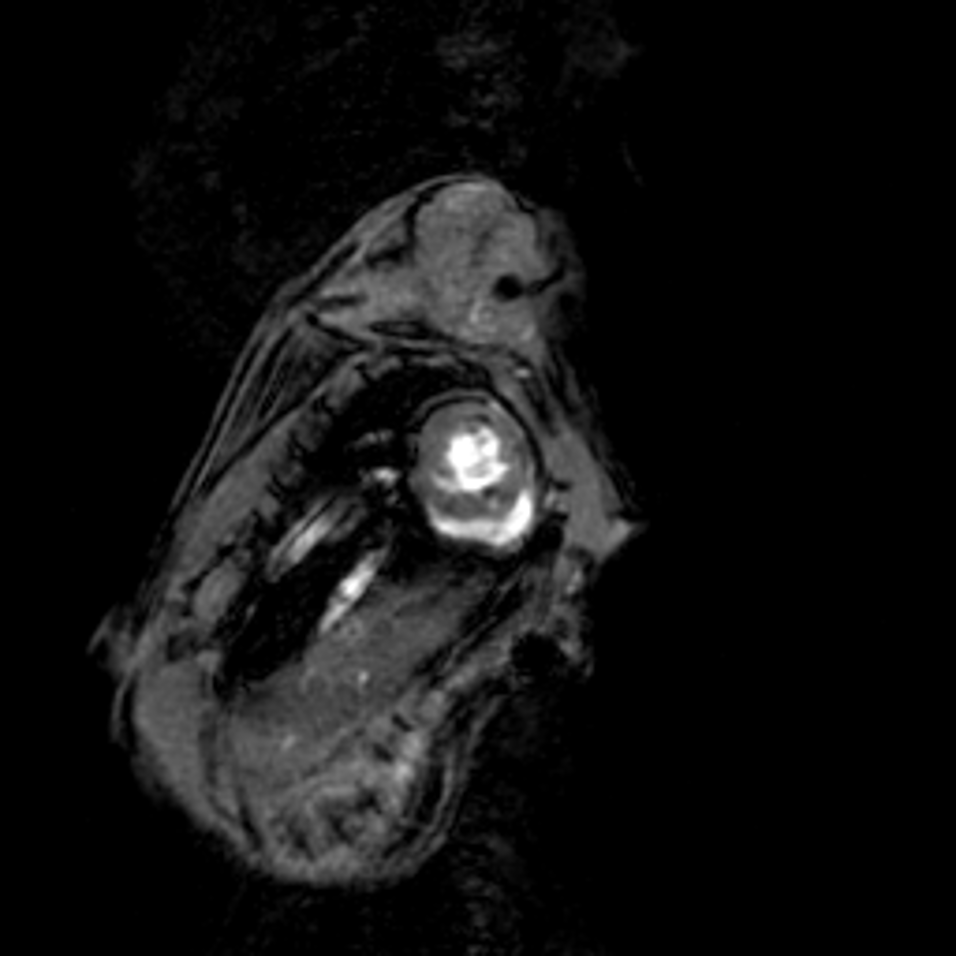
Small animal High-Intensity Focused Ultrasound (HIFU) with MRI Guidance
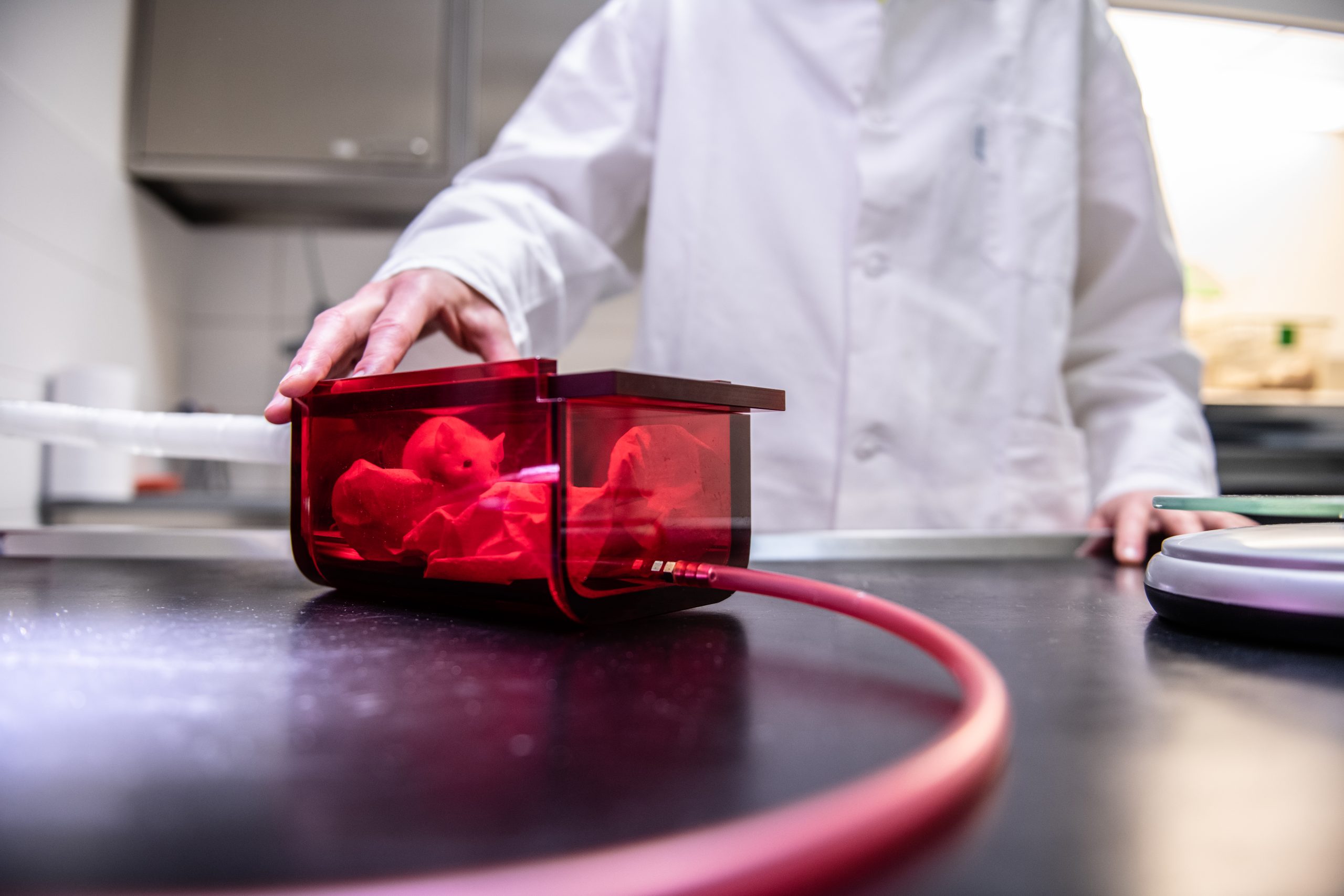
Small animal in-vivo Optical Imaging
Optical Imaging uses non ionizing radiation such as visible, ultraviolet, and infrared light to obtain detailed images of organs and tissues as well as smaller structures including cells and even molecules.
Read moreOptical Imaging is particularly useful for visualizing cells that have been engineered to express reporter genes, provided that they are located not too deep in the body. Most preclinical applications are in oncology and neuroscience research, but optical imaging is also used for cardiovascular studies and to monitor gene therapy, infectious diseases, inflammation, metabolic diseases and drug metabolism.
Available at: CUNI CAPI
Read less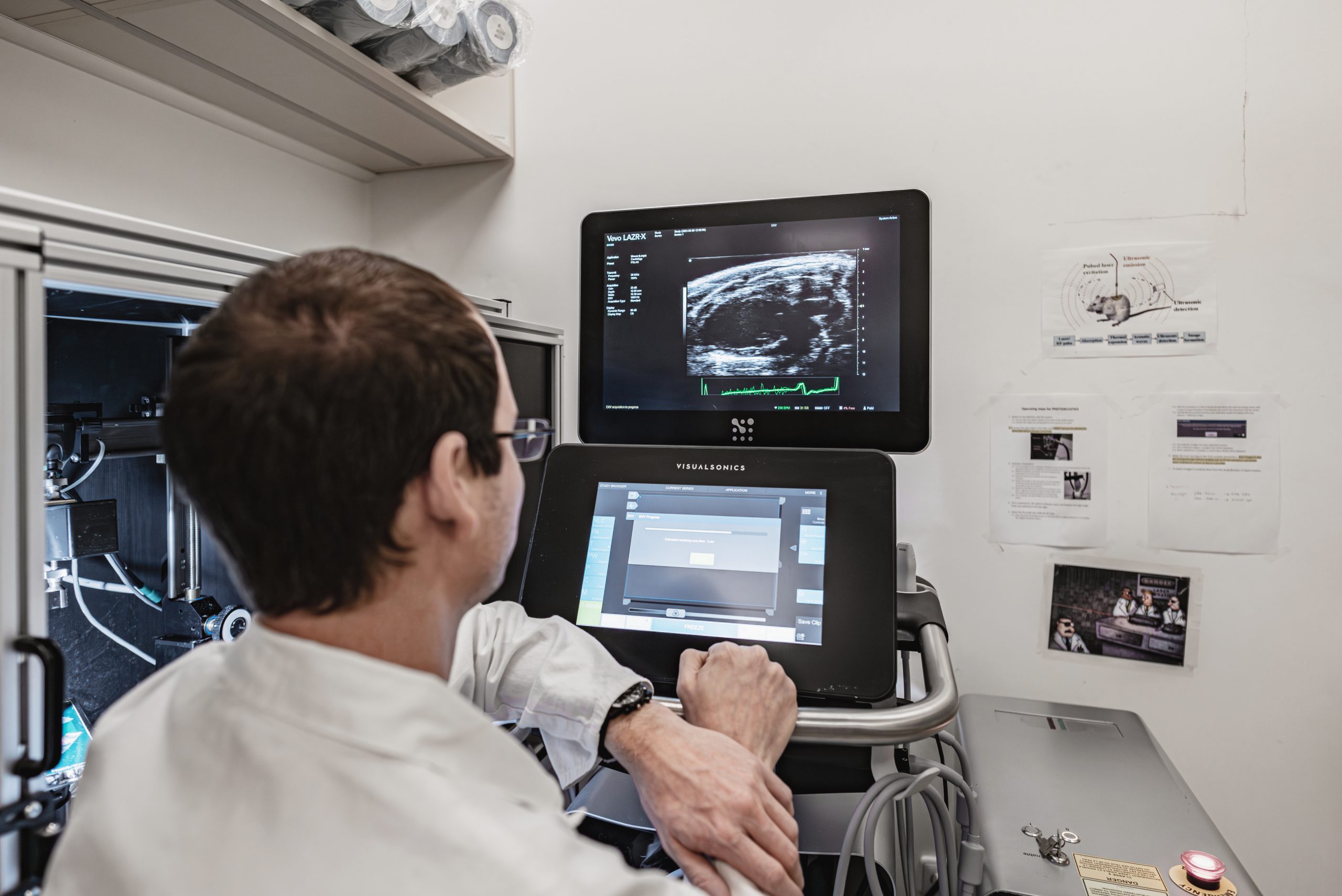
Small animal Photoacoustic Imaging
Photoacoustic imaging is an imaging modality based on the non-ionizing laser pulses, which are delivered into biological tissues and part of the energy will be absorbed and converted into heat, leading to transient thermoelastic expansion and thus wideband (i.e. MHz) ultrasonic emission.
Read moreThe generated ultrasonic waves are detected by ultrasonic transducers and then analyzed to produce images.
Available at: CUNI CAPI
Read lessHuman imaging
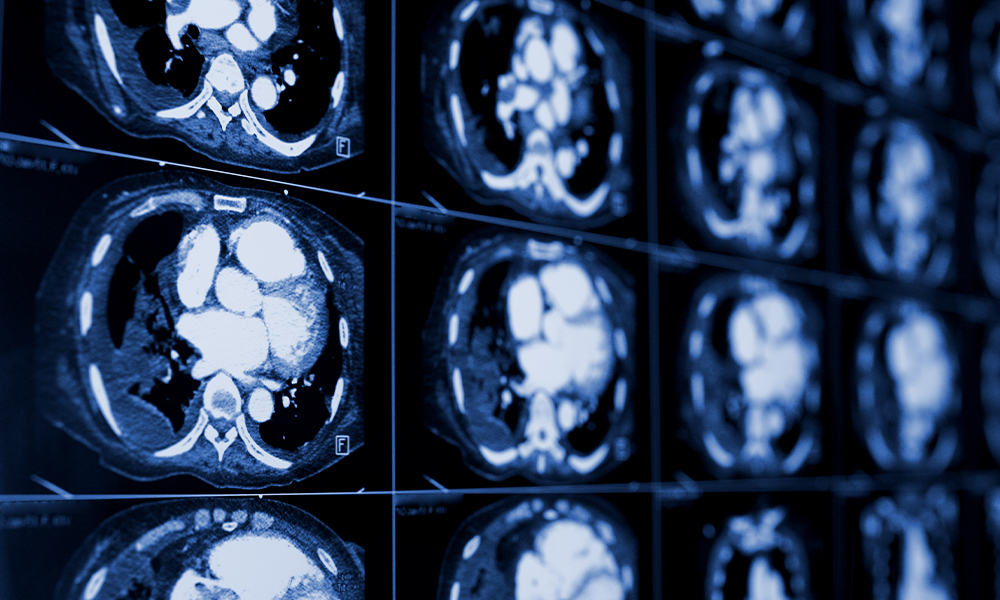
Human Magnetic Resonance Imaging (MRI) and Spectroscopy (MRS)
Magnetic resonance imaging (MRI) is a non invasive imaging technique used to obtain 2D/3D in vivo images representing anatomy and physiological processes (e.g. dynamic perfusion or dynamic changes in blood oxygenation for functional brain mapping), with high spatial and temporal resolution.
Read moreMRI scanners use strong magnetic fields (for human studies typically 1.5T to 7T), radiofrequency pulses, and field gradients to generate images of soft tissues throughout the body. Images can be enhanced with contrast agents affecting the properties of the tissue (e.g. changing relaxation times). Magnetic Resonance Spectroscopy (MRS) can be used to complement MRI in the characterization of tissues. In preclinical imaging, animal models of various pathologies, from tumors to cardiovascular, neurological or metabolic disorders, are commonly used to monitor disease development and to test the efficacy of therapeutic treatments, to develop novel diagnostic tools etc. Several quantitative imaging biomarkers can be used to monitor disease progression over time.
o MRI scanners can be used in many regimes providing several different MRI techniques. For instance: Anatomical MRI, Relaxometry, Diffusion MRI, Perfusion MRI, Functional MRI, Susceptibility weighted imaging and mapping (SWI/SWM), Chemical Exchange Saturation Transfer (CEST) and Magnetization Transfer (MT), etc.
Available at: MUNI MAFIL CEITEC
Read less
Human Electroencephalography (EEG)
The EEG is based on continuous measurement of electrical signals on the scalp over the time period (e.g. 10-30 minutes). These scalp signals represent the summation of electrical potentials in the brain and thus on the dynamic brain processes.
Read moreEEG can be used as a brain mapping technique with very high temporal resolution (in milliseconds). Beside the analysis of scalp signals, high-density EEG recordings can be used to mathematical reconstruction of the sources in the brain, i.e. to localise brain regions responsible for observed changes in electrical activity. The method can be used to study a healthy brain or to diagnose and study specific disease (e.g. epilepsy).
Available at: MUNI MAFIL CEITEC
Read less
Human Non-invasive Brain Stimulation (NIBS)
The methods for non-invasive brain stimulation are very useful tools to study brain functions and complementary tools to standard imaging/mapping techniques like MRI, EEG, PET. It enables to modify the functional properties of specific brain regions (e.g. to excite or inhibit the region/function) or the properties of connections between brain regions.
Read moreThe stimulation can be done by magnetic pulses ( transcranial magnetic stimulation – TMS) or by electric current (tDCS/tACS = transcranial direct/alternating current stimulation). These methods are typically used in combination with MRI or EEG measurement (before or/and after stimulation).
Available at: MUNI MAFIL CEITEC
Read lessImage analysis and development of new methods
Image analysis and development of new methods – image analysis using freely available, commercially available as well as custom software packages; development of tailor-made image acquisition, processing and analysis algorithms for various types of imaging techniques; 3D reconstruction of imaged specimens with high spatial resolution; visualization of results; analysis of time series of images; development of custom-made software implementations.